Wind-Optimal Cruise Airspeed Mode for Flight Management Systems (FMS)
Aerospace
Wind-Optimal Cruise Airspeed Mode for Flight Management Systems (FMS) (TOP2-319)
A new energy-efficient speed mode for FMS
Overview
Energy-efficient flight is especially important for commercial viability of aviation. Even a small amount of fuel savings - 5% per flight - can be significant because of the scale of operations, with tens of thousands of flights per day in the US alone. Energy efficient flight planning is even more important for electric Vertical Takeoff and Landing (eVTOL) aircraft with battery powered distributed electric propulsion (DEP) systems because the low specific energy of the lithium-ion polymer (Li-Po) batteries necessitates energy-efficient flight planning. NASA’s Ames Research Center has developed a novel speed mode for FMSs, a wind-optimal airspeed mode that optimizes route planning for minimum-energy usage based on actual and predicted wind conditions. Using real-time computation, an FMS can continuously update the wind-optimal airspeed target and try to maintain the optimal airspeed for current flight conditions for the entire cruise portion of the flight.
The Technology
The novel approach for optimizing airspeed for both actual and predicted wind conditions in electric Vertical Takeoff and Landing (eVTOL) aircraft with Distributed Electric Propulsion (DEP) systems includes the process of creating a lookup table for wind‐optimal airspeed as a function of wind magnitude, considering the direction of the wind relative to the cruise segment, considering the cruise altitude for an aircraft type, and incorporating the wind-optimal airspeed lookup table in the performance database for real‐time access by the Flight Management Systems (FMS) to predict wind-optimal airspeed at waypoints of the flight plan. The target wind‐optimal airspeed is updated in real-time throughout the cruise portion of a flight.
In a test of the wind-optimal airspeed targeting technique using a multi-rotor aircraft model, results obtained show benefits of flying at the wind‐optimal cruise airspeed compared to the best‐range airspeed. In headwind conditions, energy consumption was reduced by up to 7.5%, and flight duration was reduced by up to 28%. Under uncertain wind magnitudes, flying at wind-optimal airspeed offered lower variability and higher predictability in energy consumption than flying at best‐range airspeed.
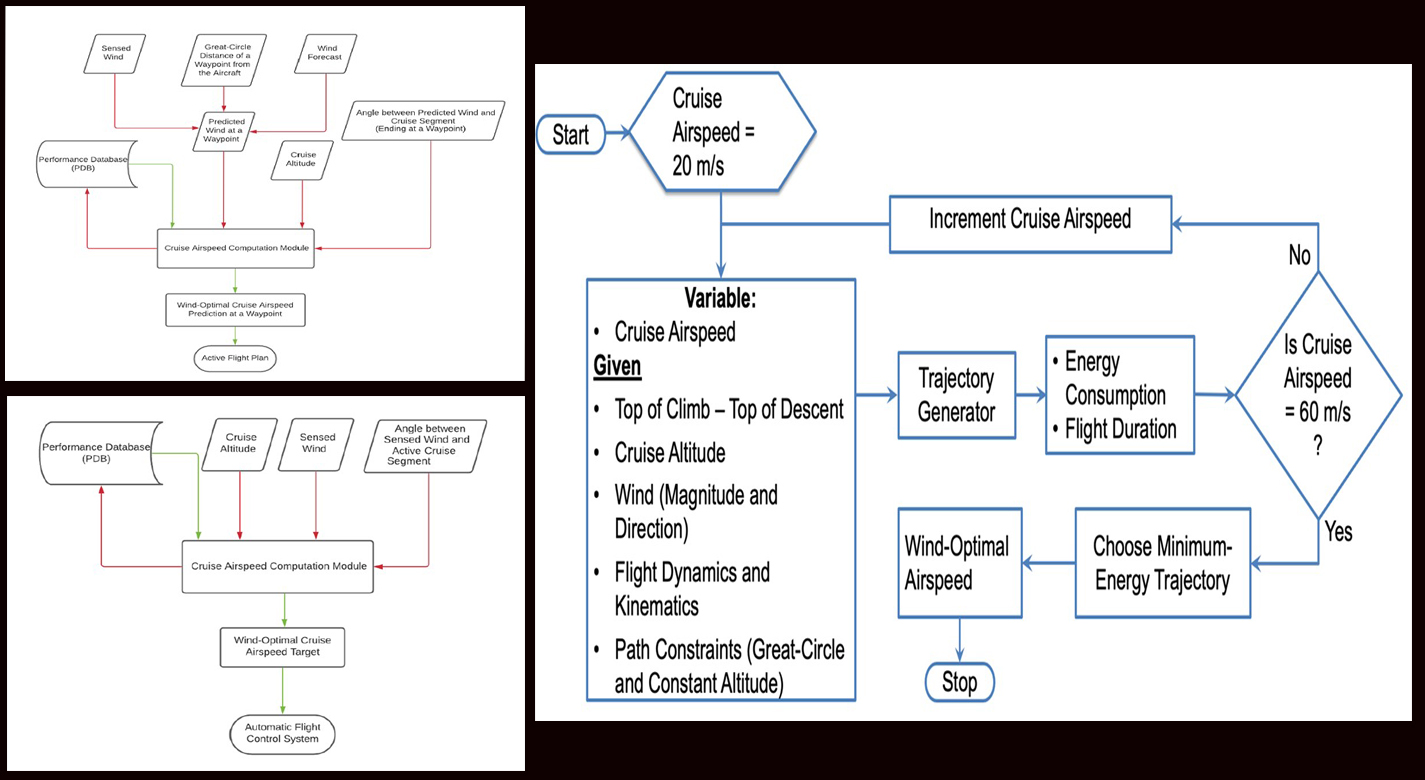
Benefits
- Energy efficient flight for eVTOL aircraft with Distributed Electric Propulsion (DEP) systems
- Reduced energy consumption of up to 7.5%
- Reduced fight duration of up to 28%
- Lower variability and higher predictability in energy consumption even under wind uncertainty
- Also useful for commercial, regional, and business jet aircraft types
- Incorporation of wind-optimal airspeed mode in the FMS enables the pilot to select and fly the aircraft at the wind-optimal airspeed autonomously
Applications
- Avionics Industry (Original Equipment Manufacturer): - FMS - Performance Management System (PMS) - Electronic Flight Bag (EFB
- Urban Air Mobility (UAM)/Advanced Air Mobility (AAM): - Provider of Services for UAM/AAM - UAM/AAM Operators
- Unmanned Aircraft System Traffic Management (UTM): - UAS Service Supplier (USS) - UAS Operators
- Aircraft manufacturing companies: - Electric Vertical Take-Off and Landing (EVTOL) aircraft - Unmanned Aircraft Systems (UAS) - Jet aircraft, turbo-prop aircraft, gas-electric hybrid aircraft and aircraft using alternative sources of power such as hydrogen
Technology Details
Aerospace
TOP2-319
ARC-18789-1
https://arc.aiaa.org/doi/10.2514/6.2022-0262
Similar Results

Fixed Wing Angle eVTOL
While previous eVTOLs often require a near 90° wing tilt to position propellers in an optimal location to generate vertical force for takeoff, NASA has taken a very different approach. NASA's design instead uses a slight wing angle and large flaps designed to deflect slipstream generated by the propellers to create a net positive force in the vertical direction, all while preventing forward movement. This unique configuration allows for takeoff and landing operations without the need for near 90° wing tilt angles. After takeoff, the transition to forward flight only requires a slight change in attitude of the vehicle and retraction of the flaps. Similar solutions require large changes in attitude to accomplish this transition which is often undesirable, especially for air taxi operations that involve passengers.
Given the effectiveness of this configuration for generating upward force, the requirement for wing angle tilt has been reduced from near 90° to approximately 15° during takeoff. Further iterations may reduce this requirement even further to 0°. By eliminating the need for near 90° wing tilt, NASA's eVTOL design removes the need for mechanisms to perform active tilting of the wings or rotors, reducing system mass and thereby improving performance. Flaps represent the only components that require actuation for takeoff and landing operations.
Innovators at NASA leveraged the Langley Aerodrome 8 (LA-8), a modular testbed vehicle that allows for rapid prototyping and testing of eVTOLs with various configurations, to design and test this novel concept.

Rapid Aero Modeling for Computational Experiments
RAM-C interfaces with computational software to provide test logic and manage a unique process that implements three main bodies of theory: (a) aircraft system identification (SID), (b) design of experiment (DOE), and (c) CFD. SID defines any number of alternative estimation methods that can be used effectively under the RAM-C process (e.g., machine learning techniques, regression, neural nets, fuzzy modeling, etc.). DOE provides a statistically rigorous, sequential approach that defines the test points required for a given model complexity. Typical DOE test points are optimized to reduce either estimation error or prediction error. CFD provides a large range of fidelity for estimating aircraft aerodynamic responses. In initial implementations, NASA researchers “wrapped” RAM-C around OVERFLOW, a NASA-developed high-fidelity CFD flow solver. Alternative computational software requiring less time and computational resources could be also utilized.
RAM-C generates reduced-order aerodynamic models of aircraft. The software process begins with the user entering a desired level of fidelity and a test configuration defined in terms appropriate for the computational code in use. One can think of the computational code (e.g., high-fidelity CFD flow solver) as the “test facility” with which RAM-C communicates with to guide the modeling process. RAM-C logic determines where data needs to be collected, when the mathematical model structure needs to increase in order, and when the models satisfy the desired level of fidelity.
RAM-C is an efficient, statistically rigorous, automated testing process that only collects data required to identify models that achieve user-defined levels of fidelity – streamlining the modeling process and saving computational resources and time. At NASA, the same Rapid Aero Modeling (RAM) concept has also been applied to other “test facilities” (e.g., wind tunnel test facilities in lieu of CFD software).

Active Turbulence Suppression System for Electric Vertical Take-Off and Landing (eVTOL) vehicles
The Active Turbulence Suppression (ATS) system for electric Vertical Take-Off and Landing (eVTOL) vehicles employ existing lifting propellers to dampen instabilities during flight, such as Dutch-roll oscillations and other gust-induced oscillations. When a roll angle of an eVTOL aircraft has deviated or is about to deviate from a current stable aircraft state to an undesirable, unstable, and oscillating aircraft state, the ATS system queries a turbulence suppression database that stores a set of propeller speed profiles for mitigation a deviation of a given roll angle for a particular aircraft with specified propellers. Using this data, the eVTOL flight controller adjusts the speed of the propellers for a certain duration of time, according to the propeller speed profiles for mitigating the deviation. In models of aircraft with adjustable propeller angles, the database includes blade angle profiles for mitigating the effects of turbulent conditions. Timing and rate of propeller activation can be pre-computed using higher order computational modeling performed with NASA’s super computing resources. Because the data is pre-computed, the use of the ATS system onboard does not require significant computing resources to implement on eVTOL vehicles. The technology, a mechanism by which existing eVTOL propellers are leveraged to suppress gust-induced oscillations enables a safe and comfortable passenger experience at low-cost and without added hardware.

Aerodynamic Framework for Parachute Deployment from Aerial Vehicle
For rapid parachute deployment simulation, the framework and methodology provided by the simulation database uses parametrized aerodynamic data for a variety of environmental conditions, air taxi design parameters, and landing system designs. The database also includes a compilation of drag coefficients, thrust and lift forces, and further relevant aerodynamic parameters utilized in the simulated flight of a proposed air taxi. The database and framework can be constructed using simulated data that accounts for oscillatory breathing of parachutes. The methodology can further employ an overset grid of body-fitted meshes to accurately capture deployment of an internally-stored parachute, as well as descent of the air taxi and deployed parachute.
The systems and methods of the disclosed technology can be utilized with existing CFD solvers in a plug-and-play manner, such that the framework can be integrated to directly improve the performance of these solvers and the machines on which they are installed. The framework itself can employ parallelization to enable distributed solution of intensive CFD simulations to build a robust database of simulated data. Further, as up to 90% of computational time is spent in the calculation of aerodynamic parameters for use in coupled trajectory equations, the framework can significantly reduce the computational costs and design time for safe landing systems for air taxis. These reductions can lead to lower costs for design processes, while enabling rapid design and testing prior to physical prototyping.

Solid-State Lithium-Sulfur Battery Tech Portfolio
The SABERS innovators developed novel lithium-sulfur designs, including sulfur-selenium on graphene cathodes, and lightweight bipolar plate stacking and packaging designs. SABERS is unique in several aspects: it deploys graphene-based manufacturing processes for the cathode and bipolar plates, and it uses a solid-state electrolyte in place of the liquid electrolyte found in other lithium-sulfur battery designs. The team has achieved energy densities over 500 W-hr/kg, and further improvements are expected. SABERS can meet the high-power requirements needed for aircraft take-off. SABERS is lightweight, safe, robust, and reliable. Furthermore, its manufacturing processes are scalable and environmentally friendly. Coin cell and pouch prototypes have been demonstrated to date. Development efforts continue and new portfolio innovations are expected. Major component technologies in SABERS include the following (as listed here and shown in the figure below).
S/Se Cathode – Sulfur/Selenium on graphene scaffold
(LAR-19556-1, LEW-20228-1)
Solid Electrolyte – Solid-state electrolyte composites
(LEW-20445-1)
Bipolar Stack – Graphene plates
(LAR-20257-1)
Li-Metal Anode
(Proprietary, under development)
Packaging
(Proprietary, under development)
Robust computational models have been developed to support the battery materials design and are available to licensees to evaluate and optimize different materials combinations and performance targets.